Nanoparticles: Small Carriers, Big Impact
- Marya Sheikh-Ahmed
- Sep 3, 2024
- 12 min read

Introduction: What are nanoparticles?
Nanoparticles (NPs) are particles with sizes ranging from 1 to 100 nanometers and can be categorized based on their properties, shapes, or sizes. Their nanoscale dimensions and large surface area make them possess unique physical and chemical characteristics. These attributes make NPs ideal for a wide range of applications, including enhancing catalysis, imaging, biomedical uses, energy research, and environmental technologies (Khan et al., 2019).
Examples of some naturally occurring nanoparticles are Silver (Ag), Gold (Au), Iron Oxide (Fe3O4), and Silica (SiO2). Ag can be found in aquatic environments and are used for their antimicrobial properties in products like plastics, paints, and cosmetics. Au are found in ore deposits and are utilized in tumor phototherapy, immunoassays, and biosensors. Fe3O4 are present in sediments and are employed in controlled drug release systems. SiO2, which can be released during volcanic eruptions or found naturally in rocks, sand, soil and water, are used as food additives, in cellular imaging, and as nanocarriers (Griffin et al., 2017).
NPs can be engineered to interact with biological systems at both the molecular and cellular levels. This precise interaction makes them highly promising tools for influencing and modulating the microbiome.
Nanocarriers
Nanocarriers (NCs) are nanoengineered, biocompatible materials or devices designed to work in combination with bioactive compounds. They play a crucial role in pharmaceutical and also in cosmetic sciences by enhancing the delivery and efficacy of therapeutic agents (Rout et al., 2018).
For instance, NCs enhance drug delivery by ensuring that antifungal medications are more effectively targeted to the infection site, improving their therapeutic impact. Their small size allows for better skin penetration as well as a controlled and sustained release of drugs, which maintains therapeutic levels longer and reduces the frequency of application. Additionally, nanocarriers improve the bioavailability of poorly soluble drugs and minimize systemic side effects by focusing the drug action more precisely on the infected areas (Keshwania et al., 2023).
Bioconjugated nanoparticulate systems are now being employed in the treatment of a range of severe and previously incurable infectious diseases, including tuberculosis, as well as chronic conditions like diabetes and various types of cancers (Rout et al., 2018).
Challenges in modulating the skin microbiome
Rising Antimicrobial Resistance
A significant challenge in skin microbiome management is the development of antimicrobial resistance. For instance, many Cutibacterium acnes strains, a common skin bacterium associated with acne, have developed resistance to major antibiotics such as erythromycin, clindamycin, doxycycline, trimethoprim/sulfamethoxazole, and tetracycline (Alkhawaja et al., 2020).
Stability and Competition of Applied Microbiota
Stabilizing applied bacteria on the skin is challenging. Despite initial topical disinfection, it is difficult to eliminate the existing subcutaneous microbiota. Consequently, new microbiota applied to the skin surface must compete with the microbiota residing in deeper skin layers, which can undermine their effectiveness (Callewaert et al., 2021).
Technical Difficulties in Probiotic Delivery
Delivering probiotics effectively presents its own set of challenges: limited concentrations, low viability in harsh environments, susceptibility to oxidative damage, challenging preservation and distribution, etc. Encapsulation technology offers a solution by enabling precise and controlled release of probiotics at varying concentrations. This method protects probiotics from harsh conditions and environmental factors such as oxygen, temperature, and light, enhancing their survival and functionality (Pandey et al., 2024).
Advances of nanotechnology in biomedical applications
To address these challenges nanotechnology has been advancing rapidly, particularly in the development of innovative drug delivery systems. Innovations in nanocarrier systems, such as nanoparticles and liposomes (Figure 1), are now engineered to specifically target pathogens or infected tissues (Zong et al., 2022).
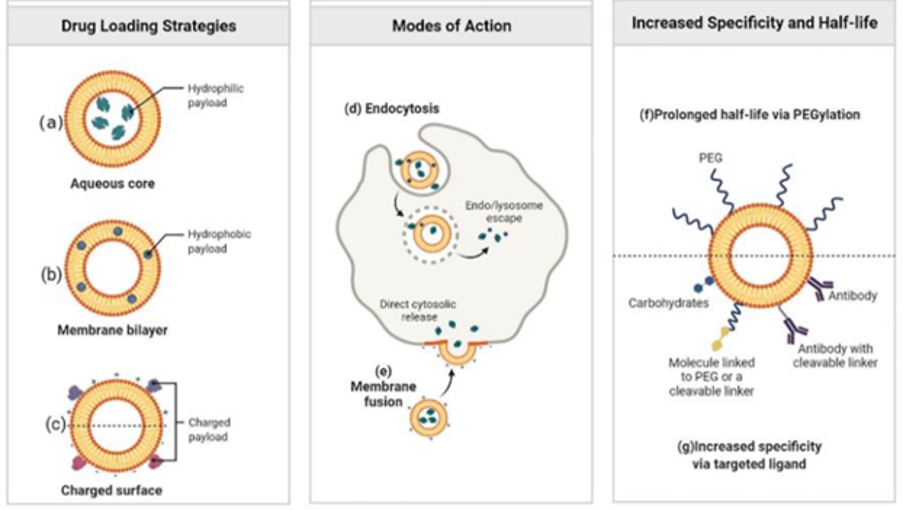
Liposomes can carry both water-soluble (Figure 1a) and fat-soluble (Figure 1b) drugs in one structure. They are biocompatible, biodegradable, low in toxicity, and cause minimal immune response. Different types of liposomes can be made positively charged, negatively charged, or neutral (Figure 1c). Liposomes interact with cells mainly through endocytosis (Figure 1d) or fusion (Figure 1e). Additionally, they can easily be modified with surface appendages allowing them to target molecules like antibodies, proteins, or enzymes to direct drugs to infection sites (Figure 1f) (Zong et al., 2022).
NP as Transdermal drug delivery systems (TDDS)
The transdermal drug delivery system is a technique that allows drugs to be absorbed through the skin. Nevertheless, the great hydrophobicity and physiology of the skin layers prevent the passive permeation of drug molecules over 500kDA, thus limiting transdermal drug diffusion. Nanoparticles have the ability to improve drug bioavailability, drug penetration and physical stability alongside providing precise dose control and targeted delivery, ensuring that drugs are released in a controlled manner and directed specifically to desired tissues or skin layers. This improved targeting helps in overcoming the skin barrier, thus enhancing treatment efficacy and reducing side effects (Palmer & DeLouise., 2016).
Drugs can penetrate the stratum corneum (SC) through two primary pathways: the transepidermal route and the transappendageal route (Figure 2).

Transepidermal route
In the transepidermal route, drugs can penetrate the skin via two pathways: the transcellular route, which is a direct path through corneocytes and lipid layers, and the intercellular route, which involves diffusing through the lipid matrix around corneocytes. Hydrophilic drugs typically use the transcellular route, while lipophilic drugs prefer the intercellular route (Barnes et al., 2021).
While lipophilic and amphiphilic molecules favour the intercellular route, the architecture of the epidermis presents a difficult path to follow causing limited permissibility. However, skin penetration enhancers (e.g. DMSO, glycols, laurocapram, etc.) can enhance drug permeation. The transcellular pathway on the other hand would be unfavorable for most drugs as they would be required to alternate hydrophilic and lipophilic regions.
Transappendagel route
The transappendageal route involves drug transport through sweat glands and hair follicles, creating channels across the SC. While these appendages cover only about 0.1% of the skin's surface and contribute minimally to drug absorption, they are crucial for ions and large polar molecules. Sweat ducts and sebaceous glands can limit drug permeation due to their hydrophilic and lipid-rich environments (Barnes et al., 2021). Notwithstanding, they benefit from accelerated transport through the skin and can serve as a reservoir for the drugs for an improved and sustained controlled localised release into skin in addition to a great proximity to the capillary vessels, facilitating systemic delivery.
How can nanoparticles be used to modulate the microbiome?
Nanocarriers as delivery systems
Delivery of antimicrobial agents
NPs can be engineered to carry antimicrobial agents like antibiotics, antimicrobial peptides, or essential oils, delivering them directly to targeted areas of the skin to combat harmful microbes. For instance, silver nanoparticles are known for their broad-spectrum antimicrobial properties, effectively inhibiting the growth of various bacteria and fungi. This makes them valuable in treating skin infections, as they can disrupt microbial cell membranes, inhibit enzyme activity, and generate reactive oxygen species (ROS) that lead to microbial death (Yin et al., 2020).
Assisting probiotic delivery
Encapsulating probiotics within protective nanocarriers acts as a physical barrier that shields them from harsh environments, such as stomach acid and bile, ensuring higher survival rates upon consumption. This encapsulation also protects probiotics from external factors like temperature and light during storage, enhancing their stability and shelf life. Additionally, nanocarriers enable the precise and controlled release of probiotics at targeted sites, maximizing their therapeutic potential. Co-encapsulation is also an option, where probiotics and other bioactive compounds are delivered together, potentially enhancing overall health benefits through synergistic effects (Pandey et al., 2024).
Targeted drug delivery
NPs can be engineered for specific cell, tissue, or location targeting by modifying their surface with ligands or antibodies, allowing for precise delivery of therapeutic agents directly to the intended site. This targeted approach significantly limits off-target effects, reducing damage to healthy cells and minimizing bystander effects. Additionally, the enhanced targeting and delivery efficiency provided by NPs enable a reduction in the required dose of the therapeutic compound, improving safety, and conserving biocompounds (Afzal et al., 2021). Hussain et al. (2018) accomplished this by conjugating a 9 amino acid oligopeptide to a porous silicon NP loaded with antibiotic targeting specifically S. aureus infected tissues.
Disruption of biofilms
A biofilm is a collection of microbial cells attached to a surface, encased in a matrix of extracellular polymeric substances (Donlan et al., 2002).
Following are few metal NPs that are known to have strong defence mechanism to combat biofilm formation;

Immunomodulation
There are four types of immunomodulatory nanosystems: organic, inorganic, biomimetic, and naturally derived. Organic nanosystems, such as liposomes and polymeric nanoparticles, are known for their biocompatibility and controlled release capabilities. Inorganic nanosystems, including metal and silica nanoparticles, offer stability and can directly interact with immune cells. Biomimetic nanosystems mimic natural biological structures, enhancing cellular uptake and immune response. Naturally derived nanosystems use compounds from natural sources, like plant extracts or microbial products, providing inherent biocompatibility and immunomodulatory properties (Khatun et al., 2023).
Case study: Probiotic-based nanoparticles for targeted microbiota modulation and immune restoration in bacterial pneumonia (Fu et al., 2022)
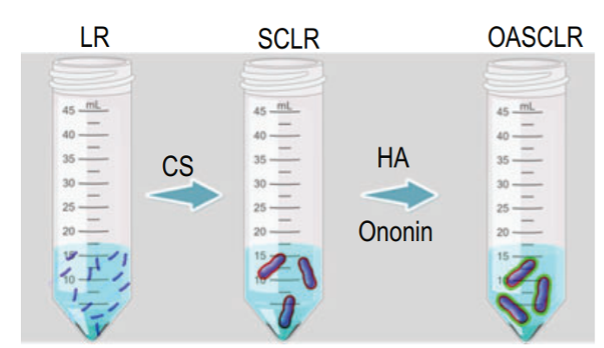
Fu et al. designed probiotic-based nanoparticles called OASCLR by coating chitosan (CS), hyaluronic acid (HA), and ononin onto living Lactobacillus rhamnosus (LR). This probiotic was chosen by virtue of its microbial competitiveness, its ability to modulate the immune response in hyperactive immunocompetent and immunocompromised hosts and its role as a modulator of the microbiome composition. To ensure the viability of LR in the lungs, the probiotic was first encapsulated in CS, known for its unique mucoadhesive properties and great biocompatibility. HA was then added as it can regulate the immune system by specifically targeting pro-inflammatory M1 macrophages via CD44 receptors. To alleviate the oxidative damage rising from the harsh conditions in the lungs, the isoflavone Ononin was added to the coating. Through ROS-scavenging, anti-inflammatory and anti-oxidant properties, ononin could enhance OASCLR’s resistance against ROS-mediated cytotoxicity and hyaluronidase degradation while also promoting the growth of LR and inhibiting pathogens. Considering the low bioactivity of LR in the ROS environment, the designed CS/HA–ononin shell could prevent LR from oxygen damage and allow OASCLR nanoparticles targeting pro-inflammatory macrophages by the interaction of HA with CD44.
These nanoparticles demonstrated over 99.97% antibacterial efficiency against common clinical pathogens. Notably, OASCLR modulated lung microbiota by reducing pathogens and enhancing the richness and diversity of probiotic and commensal bacteria. They also targeted inflammatory macrophages via CD44, alleviating excessive immune responses in hyperactive pneumonia. Additionally, OASCLR improved macrophage phagocytic function in immunocompromised pneumonia, increasing phagocytic ability from 2.61% to 12.3%. This work suggests a promising strategy for treating both hyperactive and immunocompromised bacterial pneumonia (Figure 3) (Fu et al., 2022).
Method
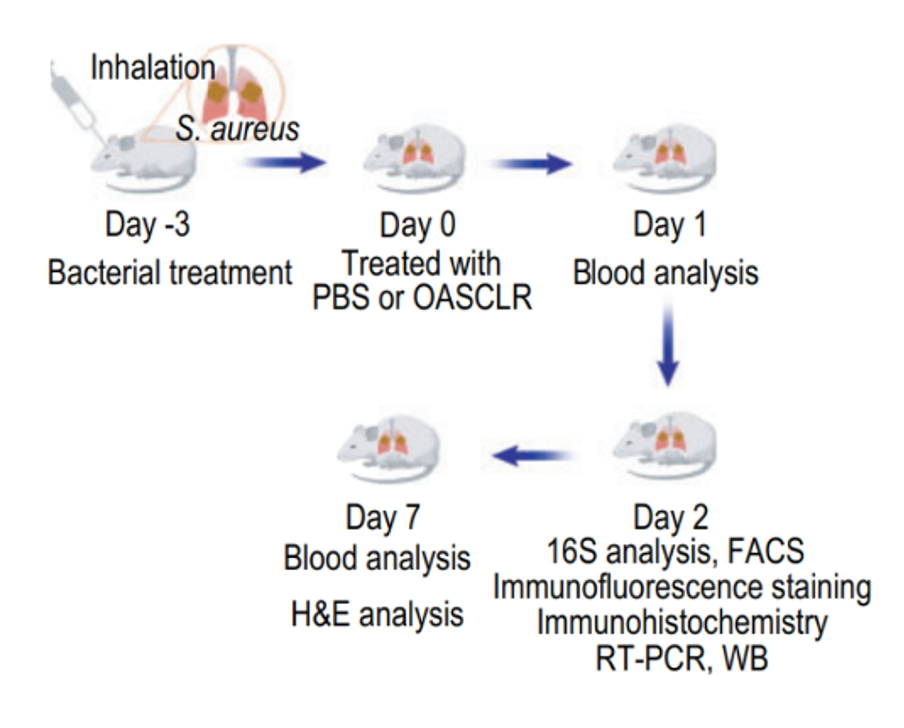
To determine whether the lung microbiome was altered following OASCLR treatment, the study established a primary pneumonia model in mice using Staphylococcus aureus (SA). The experimental design involved infecting mice with SA via nasal intubation on Day -3. The mice were then treated with either PBS as a negative control or OASCLR through non-invasive aerosol inhalation on Day 0. Blood tests were conducted on Days 1 and 7, and 16S ribosomal RNA gene sequencing was performed on Day 2 to analyze changes in the lung microbiome (Figure 4) (Fu et al., 2022).
Results: Modulation of lung microbiome by OASCLR
OASCLR group exhibited a higher Chao richness index, indicating greater bacterial species richness (Figure 5D). Further analysis showed a shift in microbiota composition, with increased Firmicutes and decreased Proteobacteria and Bacteroidota (Figure 5E). Additionally, OASCLR reduced pathogenic bacteria like Staphylococcus while boosting probiotic and commensal bacteria such as Lactobacillus and Bifidobacterium, suggesting that OASCLR effectively promotes a healthier lung microbiota (Figure 5F) (Fu et al., 2022).
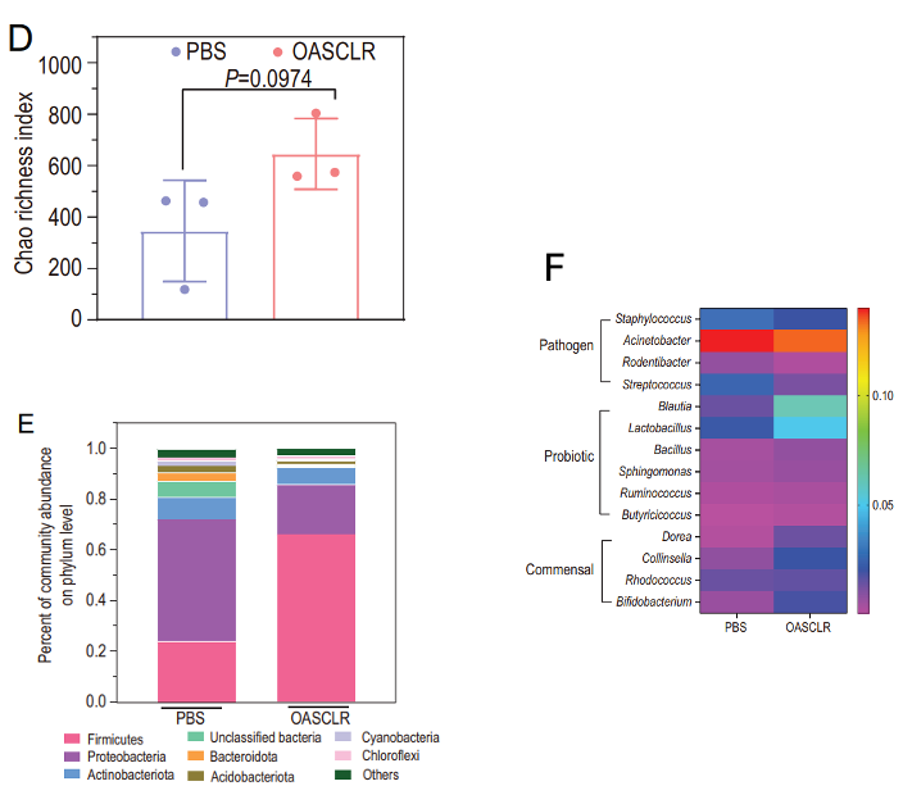
Results: Decreased inflammation
Immunofluorescence analysis revealed that OASCLR treatment reduced CD45 and TNF-α expression while slightly increasing IL-10, indicating a decrease in pro-inflammatory responses. RT-PCR analysis also showed a decreased TNF-α to IL-10 ratio in the OASCLR group compared to PBS (Figure 6). These results suggest that OASCLR effectively modulates excessive inflammation in primary bacterial pneumonia (Fu et al., 2022).
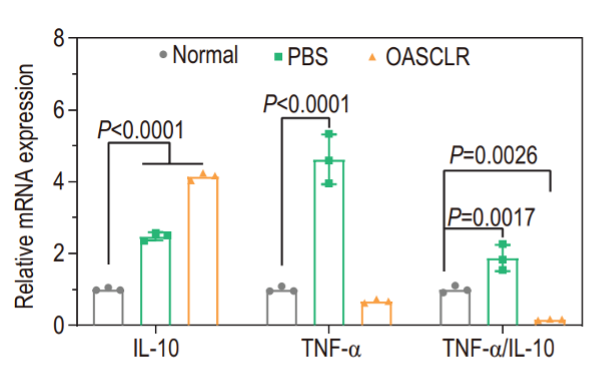
Results: Macrophage polarization
OASCLR treatment altered the immune landscape by reducing pro-inflammatory markers such as TNF-α and increasing anti-inflammatory IL-10. It also lowered the expression of CD80, a marker associated with M1 macrophages, which are typically involved in pro-inflammatory responses and tissue damage. They suggested that (This indicates that) OASCLR promotes a shift towards M2 macrophages, which are known for their anti-inflammatory properties, enhanced phagocytosis, and role in tissue repair and regeneration. This change in macrophage polarisation suggests that OASCLR helps reduce inflammation and supports tissue healing (Fu et al., 2022).
Results: Biocompatibility
The mice treated with OASCLR showed no significant tissue damage or adverse effects. Blood tests and H&E staining confirmed that OASCLR nanoparticles did not affect liver or kidney function and were safe for major organs. These findings suggest OASCLR has strong therapeutic potential for treating hyperactive immunocompetent primary pneumonia while complying to biocompatibility requirements (Fu et al., 2022).
Conclusion of the case study
OASCLR nanoparticles were reported to restore host immunity, regulate lung inflammation, and enhance macrophage phagocytosis in bacterial pneumonia. The ononin shell allows immune evasion and safe clearance, supporting clinical use. Combining probiotics with biomaterials boosts their function, making OASCLR nanoparticles a potential treatment for various diseases beyond pneumonia (Fu et al., 2022).
Consideration of NPs for microbiome modulation
Challenges in using NPs for microbiome modulation include meeting strict regulatory standards, achieving precise targeting to avoid off-target effects, and ensuring NPs stability during storage and administration. It is also important to understand the NPs degradation to ensure timely and effective drug release. These factors are critical for advancing NP-based therapies in clinical settings (Wang et al., 2017).
Conclusion: Small entities for a big future
NPs have a potential to significantly impact antimicrobial therapy and microbiome modulation despite their tiny size. NPs' unique properties, such as their ability to target specific pathogens and modulate biological systems, position them as powerful tools for advancing medical treatments. This perspective underscores the transformative possibilities of NPs in addressing current challenges in healthcare, including antibiotic resistance and precise drug delivery (Wang et al., 2017).
References
Afzal Shah, Saima Aftab, Jan Nisar, Muhammad Naeem Ashiq, Faiza Jan Iftikhar,
Nanocarriers for targeted drug delivery, Journal of Drug Delivery Science and Technology, Volume 62, 2021, 102426, ISSN 1773-2247, https://doi.org/10.1016/j.jddst.2021.102426. (https://www.sciencedirect.com/science/article/pii/S1773224721001064)
Ali SG, Ansari MA, Alzohairy MA, Alomary MN, AlYahya S, Jalal M, Khan HM, Asiri SMM,
Ahmad W, Mahdi AA, El-Sherbeeny AM, El-Meligy MA. Biogenic Gold Nanoparticles as Potent Antibacterial and Antibiofilm Nano-Antibiotics against Pseudomonas aeruginosa. Antibiotics (Basel). 2020 Feb 27;9(3):100. doi: 10.3390/antibiotics9030100. PMID: 32120845; PMCID: PMC7148532.
Alkhawaja E, Hammadi S, Abdelmalek M, Mahasneh N, Alkhawaja B, Abdelmalek SM.
Antibiotic resistant Cutibacterium acnes among acne patients in Jordan: a cross sectional study. BMC Dermatol. 2020 Nov 17;20(1):17. doi: 10.1186/s12895-020-00108-9. PMID: 33203374; PMCID: PMC7673087.
Barnes TM, Mijaljica D, Townley JP, Spada F, Harrison IP. Vehicles for Drug Delivery and
Cosmetic Moisturizers: Review and Comparison. Pharmaceutics. 2021 Nov 26;13(12):2012. doi: 10.3390/pharmaceutics13122012. PMID: 34959294; PMCID: PMC8703425.
Callewaert C, Knödlseder N, Karoglan A, Güell M, Paetzold B. Skin microbiome
transplantation and manipulation: Current state of the art. Comput Struct Biotechnol J. 2021 Jan 4;19:624-631. doi: 10.1016/j.csbj.2021.01.001. PMID: 33510866; PMCID: PMC7806958.
Donlan RM. Biofilms: microbial life on surfaces. Emerg Infect Dis. 2002 Sep;8(9):881-90. doi:
10.3201/eid0809.020063. PMID: 12194761; PMCID: PMC2732559.
Fu J, Liu X, Cui Z, Zheng Y, Jiang H, Zhang Y, Li Z, Liang Y, Zhu S, Chu PK, Yeung KWK,
Wu S. Probiotic-based nanoparticles for targeted microbiota modulation and immune restoration in bacterial pneumonia. Natl Sci Rev. 2022 Oct 16;10(2):nwac221. doi: 10.1093/nsr/nwac221. PMID: 36817841; PMCID: PMC9935993.
Griffin S, Masood MI, Nasim MJ, Sarfraz M, Ebokaiwe AP, Schäfer KH, Keck CM, Jacob C.
Natural Nanoparticles: A Particular Matter Inspired by Nature. Antioxidants (Basel). 2017 Dec 29;7(1):3. doi: 10.3390/antiox7010003. PMID: 29286304; PMCID: PMC5789313.
Hussain, S., Joo, J., Kang, J., Kim, B., Braun, G.B., She, Z.-G., Kim, D., Mann, A.P., Mölder, T., Teesalu, T., Carnazza, S., Guglielmino, S., Sailor, M.J., Ruoslahti, E., 2018.
Antibiotic-loaded nanoparticles targeted to the site of infection enhance antibacterial efficacy. Nat Biomed Eng 2, 95–103. https://doi.org/10.1038/s41551-017-0187-5
Ingle AP, Duran N, Rai M. Bioactivity, mechanism of action, and cytotoxicity of copper-based
nanoparticles: a review. Appl Microbiol Biotechnol. 2014 Feb;98(3):1001-9. doi: 10.1007/s00253-013-5422-8. Epub 2013 Dec 5. PMID: 24305741.
Jardeleza C, Rao S, Thierry B, Gajjar P, Vreugde S, Prestidge CA, Wormald PJ.
Liposome-encapsulated ISMN: a novel nitric oxide-based therapeutic agent against Staphylococcus aureus biofilms. PLoS One. 2014 Mar 21;9(3):e92117. doi: 10.1371/journal.pone.0092117. PMID: 24658315; PMCID: PMC3962386.
Keshwania P, Kaur N, Chauhan J, Sharma G, Afzal O, Alfawaz Altamimi AS, Almalki WH.
Superficial Dermatophytosis across the World's Populations: Potential Benefits from Nanocarrier-Based Therapies and Rising Challenges. ACS Omega. 2023 Aug 22;8(35):31575-31599. doi: 10.1021/acsomega.3c01988. PMID: 37692246; PMCID: PMC10483660.
Khan, Ibrahim & Saeed, Khalid & Khan, Idrees. (2019). Nanoparticles: Properties,
Applications and Toxicities. Arabian Journal of Chemistry. 12. 908-931. 10.1016/j.arabjc.2017.05.011.
Khatun S, Putta CL, Hak A, Rengan AK. Immunomodulatory nanosystems: An emerging
strategy to combat viral infections. Biomater Biosyst. 2023 Jan 30;9:100073. doi: 10.1016/j.bbiosy.2023.100073. PMID: 36967725; PMCID: PMC10036237.
Palmer BC, DeLouise LA. Nanoparticle-Enabled Transdermal Drug Delivery Systems for
Enhanced Dose Control and Tissue Targeting. Molecules. 2016 Dec 15;21(12):1719. doi: 10.3390/molecules21121719. PMID: 27983701; PMCID: PMC5639878.
Pandey, R.P., Gunjan, Himanshu et al. Nanocarrier-mediated probiotic delivery: a systematic
meta-analysis assessing the biological effects. Sci Rep 14, 631 (2024). https://doi.org/10.1038/s41598-023-50972-x
Rout GK, Shin HS, Gouda S, Sahoo S, Das G, Fraceto LF, Patra JK. Current advances in
nanocarriers for biomedical research and their applications. Artif Cells Nanomed Biotechnol. 2018;46(sup2):1053-1062. doi: 10.1080/21691401.2018.1478843. Epub 2018 Jun 7. PMID: 29879850.
Trivedi R, Upadhyay TK, Kausar MA, Saeed A, Sharangi AB, Almatroudi A, Alabdallah NM,
Saeed M, Aqil F. Nanotechnological interventions of the microbiome as a next-generation antimicrobial therapy. Sci Total Environ. 2022 Aug 10;833:155085. doi: 10.1016/j.scitotenv.2022.155085. Epub 2022 Apr 6. PMID: 35398124.
Wang L, Hu C, Shao L. The antimicrobial activity of nanoparticles: present situation and
prospects for the future. Int J Nanomedicine. 2017 Feb 14;12:1227-1249. doi: 10.2147/IJN.S121956. PMID: 28243086; PMCID: PMC5317269.
Yin IX, Zhang J, Zhao IS, Mei ML, Li Q, Chu CH. The Antibacterial Mechanism of Silver
Nanoparticles and Its Application in Dentistry. Int J Nanomedicine. 2020 Apr 17;15:2555-2562. doi: 10.2147/IJN.S246764. PMID: 32368040; PMCID: PMC7174845.
Zong TX, Silveira AP, Morais JAV, Sampaio MC, Muehlmann LA, Zhang J, Jiang CS, Liu SK.
Recent Advances in Antimicrobial Nano-Drug Delivery Systems. Nanomaterials (Basel). 2022 May 29;12(11):1855. doi: 10.3390/nano12111855. PMID: 35683711; PMCID: PMC9182179.
Comments